Atomic quantum processors make their debut
Two research groups demonstrate quantum algorithms using neutral atoms as qubits. Tim Wogan reports
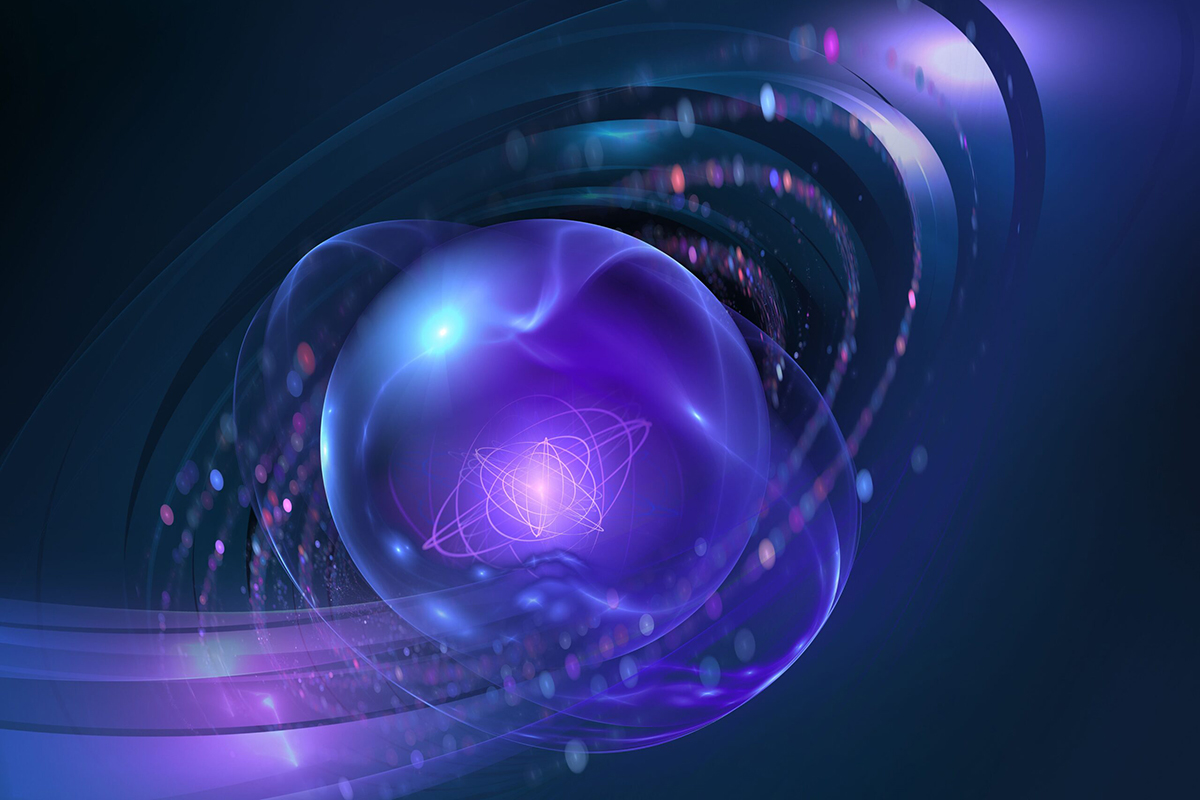
The first quantum processors that use neutral atoms as qubits have been produced independently by two US-based groups. The result offers the possibility of building quantum computers that could be easier to scale up than current devices.
Two technologies have dominated quantum computing so far, but they are not without issues. Superconducting qubits must be constructed individually, making it nearly impossible to fabricate identical copies, so the probability of the output being correct is reduced – causing what is known as “gate fidelity”. Moreover, each qubit must be cooled close to absolute zero. Trapped ions, on the other hand, have the advantage that each ion is guaranteed to be indistinguishable by the laws of quantum mechanics. But while ions in a vacuum are relatively easy to isolate from thermal noise, they are strongly interacting and so require electric fields to move them around.
In 2016, Mikhail Lukin at Harvard University and colleagues at the Massachusetts Institute of Technology (MIT) and, independently, researchers at the Institut d’Optique in Paris, unveiled a third platform – storing quantum information in neutral atoms that they manipulate using optical tweezers. By using a laser to excite the atoms to a highly ionized Rydberg state, the teams were able to entangle them with other atoms, allowing gate operations to be performed before the output of the gate was stored stably once again. However, nobody had previously demonstrated a full quantum circuit using neutral atoms.
In the latest research, two groups have managed to store quantum information in the hyperfine states of alkali atoms: rubidium for a team led by Lukin, and caesium for a team led by Mark Saffman of the University of Wisconsin-Madison together with scientists from the quantum firms ColdQuanta and Riverlane. According to Dolev Bluvstein, a colleague of Lukin at Harvard, these hyperfine states have several advantages. “If you have two atoms in a hyperfine state next to each other, because they’re so robust they don’t do anything,” he says. “So if we want to entangle two atoms on demand, we simultaneously try to excite both of them to the Rydberg state. These Rydberg states are strongly interacting, and that allows us to entangle the atoms really quickly. Then we come back down to the hyperfine state, where the entangled atoms are robust to the optical tweezer.”
The Harvard-MIT group used this robustness to physically separate the entangled atoms without causing them to decohere so that they lose their quantum information (Nature 604 451). When each atom arrives at its destination, another pulse from the laser entangles it with the next atom. This enables the group to perform non-local quantum gate operations without needing photonic or atomic links to move entanglement around the circuit. The researchers used this protocol to execute several programs. Notably, they prepared a “logical qubit” made up of seven physical qubits that could encode information in an error-resistant fashion.
Bluvstein notes that entangling multiple logical qubits would be much simpler in his team’s system than in alternatives that use static qubits. Such flexibility, Bluvstein adds, should allow researchers to perform quantum error correction and entanglement between logical qubits “in a way that simply is not possible with superconducting qubits or trapped ions”.
Precision control
The Wisconsin group took a different tack. Instead of physically moving their atoms, they used precision-controlled laser light to manipulate the atoms’ Rydberg excitations and transfer entanglement around the lattice (Nature 604 457). “Imagine you have three qubits in a line,” Saffman explains. “I’m going to take two laser beam spots and illuminate the left-most one and the centre one. They get excited to the Rydberg state, they interact, they become entangled.” The next step, Saffman continues, is to move the laser beams to illuminate the centre atom and the right one that was previously inactive and excite both to the Rydberg state. In this fashion, he concludes, “the laser beams are controlling the gate operations but the actual linking mechanisms are the atomic Rydberg interactions”.
Saffman’s group demonstrated the power of their scheme by producing six-atom states known as Greenberger-Horne-Zeilinger states, which are sometimes termed Schrödinger-cat states because they have the strongest possible non-local correlations of all multi-particle quantum states. The Wisconsin team also showed that their system could act as a quantum simulator by performing various quantum phase estimation problems, such as estimating the energy of a hydrogen molecule.
By keeping the atoms static, the Wisconsin team could link sequential gate operations more quickly than the Harvard-MIT group, albeit with some loss in flexibility. However, the Harvard-MIT group’s approach allowed them to perform multiple gate operations in parallel, thereby achieving a clock speed higher than the Wisconsin group. “Highly parallel operations with arbitrary non-local connectivity are central for building large-scale quantum machines and this is what our work demonstrates for the first time,” explains Lukin. Saffman adds that the two approaches could be combined into a single, more powerful system. “But right now they’re two fascinating examples of the multiplicity of approaches one can take,” he adds.